Seismic Reflection
Basic Concept
Seismic-reflection methods are active-source geophysical methods that were developed for oil and gas exploration applications. More recently, seismic-reflection methods have seen greater use as near-surface investigatory tools due to technological advances that increase their ability to produce high-resolution, two-dimensional images of the subsurface. Seismic-reflection methods are best suited to image subsurface environments composed of approximately horizontal layers. Because the shallow subsurface is commonly stratified, seismic-reflection methods can benefit numerous near-surface environmental and hydrogeological studies.
A seismic-reflection survey employs an above-ground sound source and receiver array to, respectively, transmit sound waves and measure their reflections off acoustic discontinuities (i.e., reflecting interfaces). Depths to acoustic discontinuities, which result from acoustic impedance contrasts, are determined using a relation between seismic velocity and seismic-wave travel time. Seismic-reflection data are most commonly used to create two-dimensional subsurface cross sections that depict the depths to and structures of the reflecting interfaces that were identified in the survey.
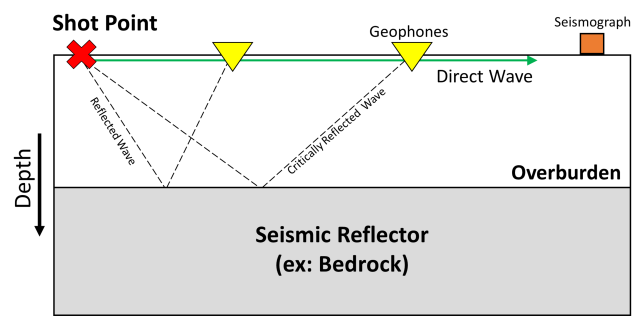
Theory
Seismic waves are waves of acoustic energy that induce oscillations within, interact with, and are influenced by subsurface earth materials. Reflection methods are most commonly concerned with the compressional (P) and shear (S) seismic body waves, which travel within the interior of geologic bodies. These waves can be differentiated by how they affect the materials through which they travel. P-waves displace material in the direction parallel to their travel direction, whereas S-wave displacement is normal (i.e., perpendicular) to their travel direction.
In homogeneous media, a seismic wave travels semispherically such that the instantaneous extent of seismically influenced material (i.e., wavefront) has a radius that increases with propagation. Waves can be described by their rays, each of which travels perpendicular to the wavefront and arrives at an acoustic interface at an angle of incidence (Θi ). This angle, which is between 0° and 90°, is formed by an arriving (i.e., incident) ray and normal to the interface.
A seismic ray travels from a surface-source point to a receiver via one of three paths. Direct rays travel just beneath the surface toward the receiver. Refracted rays arrive at reflecting interfaces (i.e., reflectors) at a critical angle of incidence (Θic ), are deflected away from normal, and travel along the bottom of the reflector. Reflected rays reach reflectors at all incident angles, and wave energy is immediately redirected to the surface (Mussett and Khan, 2000).
Seismic rays are reflected by interfaces formed by materials that have a sufficient contrast in acoustic impedance. Acoustic impedance is a measure of opposition to acoustic energy and depends upon the material’s density and seismic velocity (i.e., wave- and material-dependent seismic-wave travel speed). To a degree depending on the acoustic impedance contrast, rays are partially reflected, and the remaining portion of energy continues on toward deeper reflectors. Thus, seismic-reflection methods are able to image multilayered systems.
A true vertical cross-section of subsurface reflectors is produced by converting two-way travel times (TWT) to depths using seismic-velocity information. The TWT is the time a reflected ray takes to travel from source to receiver. The ray that reflects off a single horizontal reflector underneath the source travels the least, and the time it takes for rays to reach subsequent geophones is progressively longer. This incremental time delay (i.e., normal moveout (NMO)) is used to determine seismic velocity and layer thickness as described by Mussett and Khan (2000).
Applications
Seismic-reflection surveys employ arrays of multiple receivers (i.e., geophones) that are often equally spaced and offset to at least one side of the seismic-source location (i.e., shot point). Data may be improved by using multiple shot points, which are often offset near to, far from, and centered in between both ends of the survey line. Site-specific (i.e., wave type, energy magnitude) seismic signals can be actively transmitted into the subsurface by explosions, vibrators, accelerated-weight drops, or hammers.
Once triggered by the shot, a seismograph continuously records the magnitude and direction (i.e., positive or negative) of the seismic energy received at each geophone. The raw data record (i.e., seismogram) consists of “wiggle” traces that show variations in seismic-energy amplitude. The side-by-side comparison of these traces line up to display reflectors as a function of time, and calculations are used to determine reflector depths (Mussett and Khan, 2000).
Both compressional (P) and shear (S) waves can be useful, and the wave type chosen as signal source is done so based on site characteristics and project goals. Because of their higher seismic velocity and how they interact with materials, P-waves are able to travel through all liquids and solids. Furthermore, P-waves can penetrate coarser-grained or higher-density materials and provide lower-resolution images of the bedrock surface (Pugin and others, 2013). However, P-wave reflection at the groundwater surface is very strong.
As such, imaging layers below the water table with P-wave signals may require high-energy sources that may be unreasonable or unsafe. S-waves typically penetrate well through saturated materials and are highly resistant to absorption due to saturation. Thus, S-waves may be better suited for saturated systems, and shear-wave seismic velocity correlates well with lithology. Furthermore, S-waves often have a shorter wavelength, which allows for imaging the subsurface with a comparatively better resolution (Haines and Ellefsen, 2010).
Seismic-reflection methods are ideal for imaging horizontal strata, and resolving non-horizontal (e.g., dipping, curved, faulted, etc.) reflectors is more complex due partially to how they deviate from method assumptions. For example, dipping reflectors appear to be shallower, and faulted reflectors produce diffraction hyperbolas at their terminal ends. Though such scenarios can be dealt with in data processing using the migration correction techniques, considering ancillary geophysical, drilling, or mapping information may still be beneficial (Mussett and Khan, 2000).
The depth of investigation (DOI) depends partially on the geophone array and, generally, can be increased by increasing its lateral extent. Additionally, there can be a tradeoff between DOI and vertical resolution, as they are both affected by the frequency and wavelength of the seismic source. Vertical resolution may be improved by using shorter signal pulses of higher frequency signals, but this may decrease the DOI. Thus, estimated target depth and project goals determine field methodology and survey design (Wightman and others, 2003).
Furthermore, there exist some situations in which the seismic-reflection method may fail to detect certain features or otherwise prove to be inaccurate. Layers with a thickness less than half of the signal wavelength cannot be resolved, and interfaces with a gradational change in acoustic impedance may not produce reflections. Other complications may arise due to ambient or artificial noise sources and lateral and/or vertical velocity variations within a single geologic unit.
At late times in the seismic record, signal strength is significantly weakened, and erroneous noise or strong multiples can make true reflections difficult to identify. Though, seismic multiples (i.e., reflections off the same interface that have later arrival times) can generally be identified by their slope, which increases with each anomalous reflection. Despite being influenced by numerous factors, seismic-reflection data can be significantly improved by data processing techniques, which can produce accurate subsurface images to be applied to the following:
- Determining depth to groundwater
- Mapping bedrock surface
- Locating voids and cavities
- Estimating stratigraphy
- Determining geologic structures
- Identifying perched water or gas-filled sediments
- Coal/mineral/hydrocarbon exploration
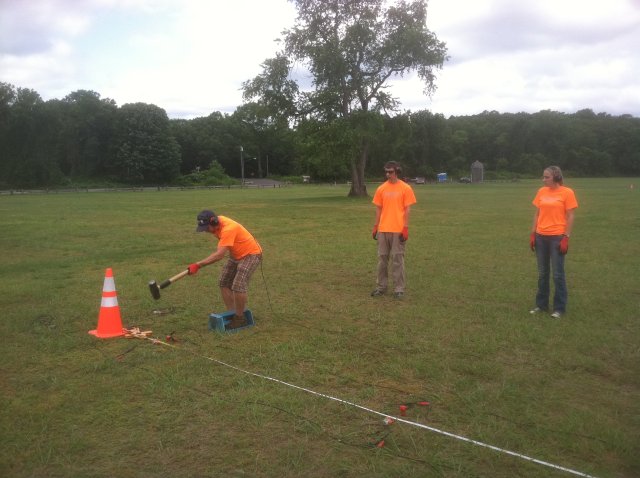
Examples/Case studies
Birkelo, B.A., Steeples, D.W., Miller, R.D., and Sophocleous, M., 1987, Seismic Reflection Study of a Shallow Aquifer During a Pumping Test: Groundwater, v. 25, no. 6, p. 703-709, doi:10.1111/j.1745-6584.1987.tb02211.x.
Abstract: Seismic reflection surveys were used to follow the drawdown in a shallow aquifer during a pumping test. Using severe analog low‐cut filters and 1/4‐m geophone spacings, 335 Hz reflections were obtained from the top of the saturated zone 2.7 m deep. The reflections moved down in time as the saturated zone dropped in response to pumping. The dominant frequency and bandwidth both dropped during pumping indicating a more diffuse reflecting boundary. Slight pullups of reflectors at specific locations on the CDP sections may indicate a higher elevation of the capillary fringe and therefore finer sediments in those locations. Other potential applications of this technique include mapping cones of depression and detecting and delineating perched‐water tables.
Bruno, P.P., Improta, L., Castiello, A., Villani, F., and Montone, P., 2010, The Vallo di Diano Fault System: New Evidence for an Active Range-Bounding Fault in Southern Italy Using Shallow, High-Resolution Seismic Profiling: Bulletin of the Seismological Society of America, v. 100, no. 2, p. 882-890, doi:10.1785/0120090210.
Abstract: Range-bounding normal faults can present significant challenges for seismic exploration. This is the case of the fault system bounding the Vallo di Diano, the largest intermountain basin in the southern Apennines seismic belt. Industry reflection profiles define the large-scale structure of the basin but barely image the shallow fault system due to unfavorable topographic and near-surface conditions along the foothills of the eastern range. We present two high-resolution (HR) wide-aperture profiles recorded at the eastern margin of the basin across unreported scarps that affect Middle–Late Pleistocene alluvial fans and slope debris. The survey is aimed at identifying possible recent faulting across these challenging terrains and at understanding the relationship between shallow structures and the master range-bounding fault at depth. Common depth point processing of wide-aperture reflection data and first-arrival travel-time tomography provide detailed images of the upper 200–300 m and sounding evidence of recent activity along previously unknown splays of the fault system. These splays dissect the Mesozoic limestone bedrock and alluvial-fan sequences, affecting their depositional pattern. Very high resolution VP and reflectivity images also give hints of possible coseismic surface faulting in Holocene colluvia. These results have relevant implications for the evaluation of the seismogenic potential of the range-bounding fault system and for seismic hazard assessment of the densely urbanized Vallo di Diano basin.
Büker, F., Green, A.G., and Horstmeyer, H., 1998, Shallow seismic reflection study of a glaciated valley: Geophysics, v. 63, no. 4, p. 1395-1407, doi:10.1190/1.1444441.
Abstract: Shallow seismic reflection data were recorded along two long (>1.6 km) intersecting profiles in the glaciated Suhre Valley of northern Switzerland. Appropriate choice of source and receiver parameters resulted in a high‐fold (36–48) data set with common midpoints every 1.25 m. As for many shallow seismic reflection data sets, upper portions of the shot gathers were contaminated with high‐amplitude, source‐generated noise (e.g., direct, refracted, guided, surface, and airwaves). Spectral balancing was effective in significantly increasing the strength of the reflected signals relative to the source‐generated noise, and application of carefully selected top mutes ensured guided phases were not misprocessed and misinterpreted as reflections. Resultant processed sections were characterized by distributions of distinct seismic reflection patterns or facies that were bounded by quasi‐continuous reflection zones. The uppermost reflection zone at 20 to 50 ms (∼15 to ∼40 m depth) originated from a boundary between glaciolacustrine clays/silts and underlying glacial sands/gravels (till) deposits. Of particular importance was the discovery that the deepest part of the valley floor appeared on the seismic section at traveltimes >180 ms (∼200 m), approximately twice as deep as expected. Constrained by information from boreholes adjacent to the profiles, the various seismic units were interpreted in terms of unconsolidated glacial, glaciofluvial, and glaciolacustrine sediments deposited during two principal phases of glaciation (Riss at >100 000 and Würm at ∼18 000 years before present).
Dehghannejad, M., Juhlin, C., Malehmir, A., Skyttä, P., and Weihed, P., 2010, Reflection seismic imaging of the upper crust in the Kristineberg mining area, northern Sweden: Journal of Applied Geophysics, v. 71, no. 4, p. 125-136, doi:10.1016/j.jappgeo.2010.06.002.
Abstract: The Kristineberg mining area is located in the western part of the Palaeoproterozoic Skellefte Ore District, one of the most important mining districts in Europe. As a part of a 3D geologic modeling project, two new reflection seismic profiles were acquired with a total length of about 20 km. One profile (HR), parallel to previous seismic profiles, was acquired using a 10 m receiver and source interval and crosses the steeply dipping structures of the Kristineberg mine. The other profile (Profile 2) runs perpendicular to all existing profiles in the area. Although the structural geology is complex, the processed seismic data reveal a series of steeply dipping to sub-horizontal reflections, some of which reach the surface and allow correlation with surface geology. Our general interpretation of the seismic images is that the Kristineberg mine and associated mineral horizon are located in the northern part of a series of steeply south dipping structures. Overall, main structures plunge to the west at about 30°–40°. Cross-dip analysis and reflection modeling were carried out to obtain the 3D orientation of the main reflections and to provide insight into the possible contribution of out-of-the-plane reflections. This helped, for example, to obtain the 3D geometry of a deep reflection that was previously interpreted as structural basement to volcanic rocks. The new reflection seismic profiles have improved our understanding of shallow geological structures in the area and in conjunction with recently acquired potential field data, magnetotelluric data and geological observations will help to refine previous 3D geologic modeling interpretations that were aimed at larger scale structures.
Haines, S.S. and Ellefsen, K.J., 2010, Shear-wave seismic reflection studies of unconsolidated sediments in the near surface: Geophysics, v. 75, no. 2, p. 1MA-Z39, doi:10.1190/1.3340969.
Abstract: We have successfully applied of SH-wave seismic reflection methods to two different near-surface problems targeting unconsolidated sediments. At the former Fort Ord, where the water table is approximately 30m deep, we imaged aeolian and marine aquifer and aquitard stratigraphy to a depth of approximately 80m. We identified reflections from sand/clay and sand/silt interfaces and we mapped these interfaces along our transects. At an aggregate study site in Indiana, where the water table is at a depth of 1to2m, we imaged stratigraphy in alluvial sand and gravel, and observe a strong reflection from the 32-m-deep bedrock surface. In both cases, we exploited the high resolution potential of SH waves, their insensitivity to water content, and the possibility of reducing Love wave contamination by working along a roadway. We accomplished our results using only sledgehammer sources and simple data processing flows.
Hunter, J.A., Pullan, S.E., Burns, R.A., Gagne, R.M., and Good, R.L., 1984, Shallow seismic reflection mapping of the overburden‐bedrock interface with the engineering seismograph—Some simple techniques: Geophysics, v. 49, no. 8, p. 1140-1395, doi:10.1190/1.1441766.
Abstract: Where unconsolidated overburden exceeds 20 m in thickness, the reflection method may be efficiently used with a 12‐channel engineering seismograph to map topography on the overburden‐bedrock interface as well as possible structure within the overburden. The two techniques we suggest are the simplest forms of reflection profiling which can be applied with a minimum amount of equipment which we suggest is a 12‐channel enhancement seismograph, a 12‐geophone array, and a hammer source. These techniques require good transmission characteristics of the overburden as well as a sharp velocity discontinuity at the overburden‐bedrock interface. For data processing and display a microcomputer is essential.
Miller, R.D., Steeples, D.W., and Brannan, M., 1989, Mapping a bedrock surface under dry alluvium with shallow seismic reflections: Geophysics, v. 54, no. 12, p. 1528-1534, doi:10.1190/1.1442620.
Abstract: Shallow seismic-reflection techniques were used to image the bedrock-alluvial interface, near a chemical evaporation pond in the Texas Panhandle, allowing optimum placement of water-quality monitor wells. The seismic data showed bedrock valleys as shallow as 4 m and accurate to within 1 m horizontally and vertically. The normal-moveout velocity within the near-surface alluvium varies from 225 m/s to 400 m/s. All monitor-well borings near the evaporation pond penetrated unsaturated alluvial material. On most of the data, the wavelet reflected from the bedrock-alluvium interface has a dominant frequency of around 170 Hz. Low-cut filtering at 24 dB/octave below 220 Hz prior to analog-to-digital conversion enhanced the amplitude of the desired bedrock reflection relative to the amplitude of the unwanted ground roll. The final bedrock contour map derived from drilling and seismic-reflection data possesses improved resolution and shows a bedrock valley not interpretable from drill data alone.
Pugin, A.J.-M., Pullan, S.E., and Duchesne, M.J., 2013, Regional hydrostratigraphy and insights into fluid flow through a clay aquitard from shallow seismic reflection data: The Leading Edge, v. 32, no. 7, p. 725-856, doi:10.1190/tle32070742.1.
Abstract: In the Montérégie region, east of Montreal, Quebec, Canada, high-resolution compressional (P-) and shear (S-) wave reflection sections were obtained using a vibratory source and three-component (3C) landstreamer data acquisition system during field programs carried out in 2009 and 2010. The work was part of a strategy to improve the knowledge and understanding of groundwater resources within the glacial and postglacial sediments in the region. The >100 line-km of seismic profiling is an excellent example of hydrogeophysics, providing detailed information on the depth to bedrock, the architecture and stratigraphy, and physical properties of the overlying sediments on both the regional and local scales (hydrostratigraphic mapping), as well as insights into fluid (water and/or gas) transport through the surface aquitard. Correlations of porosity and shear-wave velocity in the region also allow some estimation of the variation in subsurface porosities for hydrogeological modeling.
References
Birkelo, B.A., Steeples, D.W., Miller, R.D., and Sophocleous, M., 1987, Seismic Reflection Study of a Shallow Aquifer During a Pumping Test: Groundwater, v. 25, no. 6, p. 703-709, doi:10.1111/j.1745-6584.1987.tb02211.x.
Bruno, P.P., Improta, L., Castiello, A., Villani, F., and Montone, P., 2010, The Vallo di Diano Fault System: New Evidence for an Active Range-Bounding Fault in Southern Italy Using Shallow, High-Resolution Seismic Profiling: Bulletin of the Seismological Society of America, v. 100, no. 2, p. 882-890, doi:10.1785/0120090210.
Büker, F., Green, A.G., and Horstmeyer, H., 1998, Shallow seismic reflection study of a glaciated valley: Geophysics, v. 63, no. 4, p. 1395-1407, doi:10.1190/1.1444441.
Dehghannejad, M., Juhlin, C., Malehmir, A., Skyttä, P., and Weihed, P., 2010, Reflection seismic imaging of the upper crust in the Kristineberg mining area, northern Sweden: Journal of Applied Geophysics, v. 71, no. 4, p. 125-136, doi:10.1016/j.jappgeo.2010.06.002.
Haines, S.S. and Ellefsen, K.J., 2010, Shear-wave seismic reflection studies of unconsolidated sediments in the near surface: Geophysics, v. 75, no. 2, p. 1MA-Z39, doi:10.1190/1.3340969.
Hunter, J.A., Pullan, S.E., Burns, R.A., Gagne, R.M., and Good, R.L., 1984, Shallow seismic reflection mapping of the overburden‐bedrock interface with the engineering seismograph—Some simple techniques: Geophysics, v. 49, no. 8, p. 1140-1395, doi:10.1190/1.1441766.
Miller, R.D., Steeples, D.W., and Brannan, M., 1989, Mapping a bedrock surface under dry alluvium with shallow seismic reflections: Geophysics, v. 54, no. 12, p. 1528-1534, doi:10.1190/1.1442620.
Mussett, A.E., and Khan, M.A., 2000, Looking into The Earth: An Introduction to Geological Geophysics: New York, Cambridge University Press, 470 p.
Pugin, A.J.-M., Pullan, S.E., and Duchesne, M.J., 2013, Regional hydrostratigraphy and insights into fluid flow through a clay aquitard from shallow seismic reflection data: The Leading Edge, v. 32, no. 7, p. 725-856, doi:10.1190/tle32070742.1.
Wightman, W.E., Jalinoos, F., Sirles, P., and Hanna, K., 2003, Application of Geophysical Methods to Highway Related Problems: Lakewood, CO, Federal Highway Administration, Office of Bridge Technology, 742 p.